Can carbon capture take the UK beyond net zero?
Chapter 1:
From the dust of the Permian Basin
There’s not a lot going on in Scurry County, Texas. Flat, sparsely populated, dusty ranch land stretches to the horizon, with the occasional rugged plant life baking under the relentless sun and huge blue sky.
At the crossroads of Highway 180 and County Road 253 an oil field equipment supplier sits opposite a former Baptist church.
It’s just one county in the expanse of 220,000 square kilometres that makes up the Permian Basin. Spanning across West Texas and New Mexico, the basin is one of the most productive regions for crude oil extraction in the world, generating as many as 4 million barrels on average every single day in 2019.
It’s an unlikely place to go looking for a technology with the potential to decarbonise energy and industries on a massive scale, but in 1972 Scurry County was home to the first ever use of carbon dioxide (CO2) in a process called ‘enhanced oil recovery’. By injecting CO2 hundreds of metres below the earth’s surface, oil companies could flush leftover oil through rock formations to their wells and pump it from the ground.
This technology has long helped make the Permian Basin’s oil fields, some 7,000 of them, among the most fruitful in the world. But the approach of taking CO2 and trapping it underground could have a much bigger role to play in an era beyond oil wells.
Rather than being used to extract fossil fuels, injecting CO2 permanently into existing geological formations offers a means of reducing the amount of emissions that enter the earth’s atmosphere and, ultimately, reducing the impact of climate change.
It’s a very different use of the process, but by offering a proven way to capture and store CO2 safely and permanently, it opens the door to what could be one of the most important innovations of the 21st century: carbon capture, usage and storage (CCUS).
What is carbon capture?
CCUS is, in simple terms, the process of capturing CO2 from a source, such as a power station or factory, and preventing it from being released into the atmosphere. It can then be used to make new products or in industrial processes, but the vast majority will need to be stored permanently.
Report after report, from many different governments and institutions, has highlighted how vital CCUS will be in reducing CO2 levels from sources of emissions – in particular industrial processes, such as power generation or cement and steel manufacturing.
Beyond just capturing carbon dioxide, the technology has the potential to deliver negative emissions.
CCUS is needed on a massive scale if the world is going to meet the Paris Agreement’s climate targets of keeping global temperatures within 1.5oC of pre-industrial levels, to avert catastrophic climate change. But beyond just capturing the CO2 that humans produce, the technology has the potential to deliver negative emissions, taking CO2 out of the atmosphere and storing it in a way that means global warming can be slowed or even, eventually, reversed.
But at a time when renewable energy and low carbon alternatives are increasing, why is there still a need for carbon capture?
The need for CCUS
Decarbonisation is a race against time. The UK has set 2050 as the year in which it aims to reach net zero, a key step towards meeting the goals of the five-year-old Paris Agreement.
What net zero actually means is different from cutting all emissions from factories, transport and all the other processes that we depend upon, down to zero. Instead, net zero means that the amount of greenhouse gas (GHG), including CO2, emitted and removed are balanced.
For countries, cities and companies around the world this means as well as making efforts to reduce emissions, an equal amount of carbon must be verifiably captured, absorbed or offset. The result is that the carbon emitted is the same as the amount removed, so it does not increase carbon levels in the atmosphere. Carbon contributions are effectively zero.
But what if more carbon could be absorbed, offset or captured from the atmosphere than is emitted? This is where negative emissions come in.
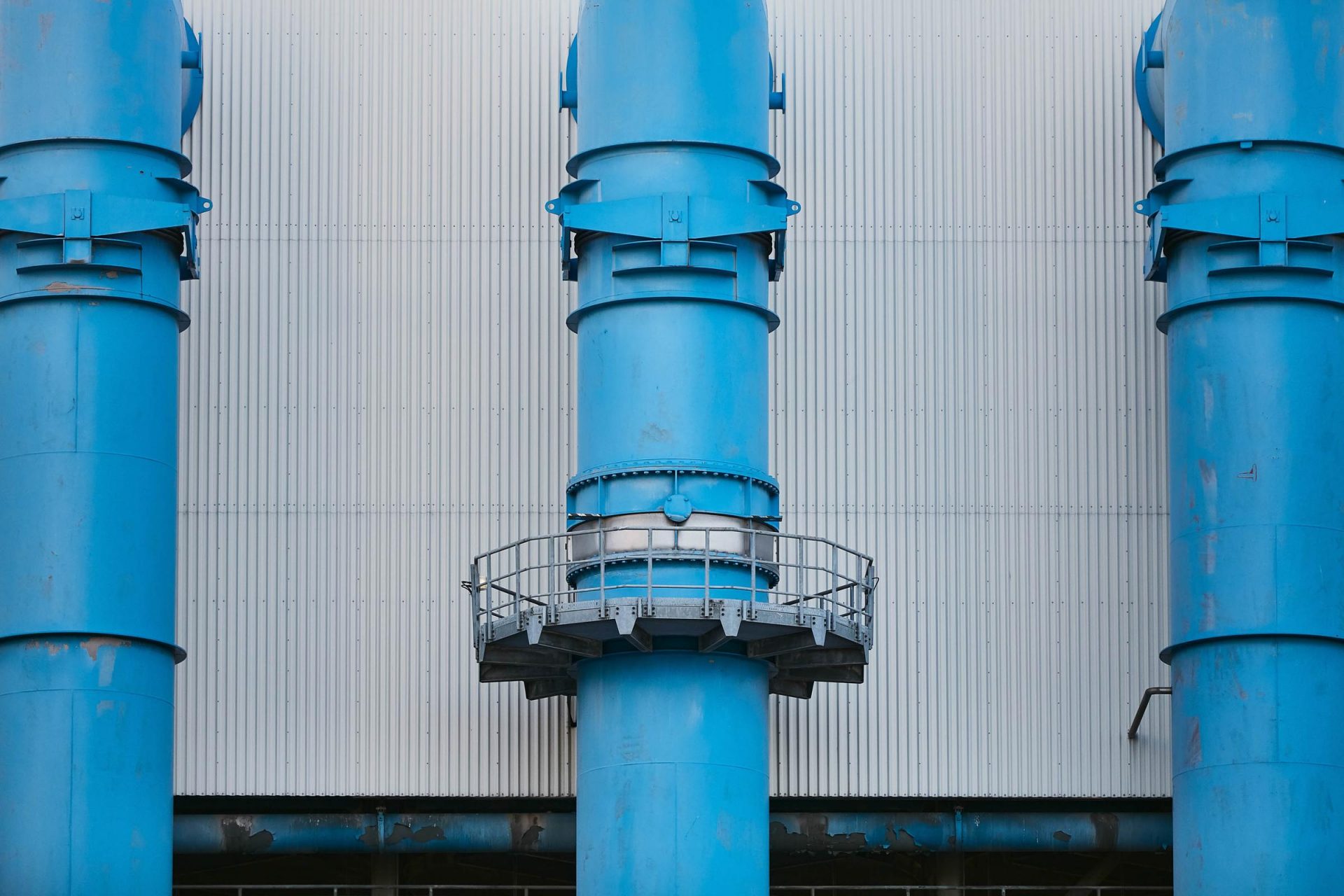
There are a variety of ways to take more carbon from the atmosphere than is emitted. Planting forests is an obvious example – trees absorb CO2 through photosynthesis and release oxygen. But there are human-engineered ways of removing CO2 from the atmosphere. One of the most crucial according to the UK Committee on Climate Change is bioenergy with carbon capture and storage – or BECCS.
Bioenergy replaces fossil fuels with biomass, made of organic matter that absorbs CO2 from the atmosphere as it grows. When BECCS technology is applied, as the fuel is combusted, the same CO2 is then captured, transported and stored permanently underground. The amount of CO2 in the atmosphere has decreased and the carbon-dense fossil fuels below the earth’s crust remain put.
The concept behind carbon capture and negative emissions has existed for decades, but now it is finally on the verge of becoming a reality on a scale that can make a difference. Industries around the world are already experimenting and trialling various forms of CCUS. The urgent need for countries to rapidly reduce their CO2emissions is creating a new imperative to implement the technology in a way that can bring about true carbon neutrality and help to prevent catastrophic climate change.
It will take the technology far away from the dust of the Permian Basin and beyond the energy systems that depend on fossil fuels. It offers a future built on science, technology and knowledge around energy, emissions and CO2 itself.
Chapter 2:
What is carbon?
If you asked a scientist to choose one of all the elements known to humans, that could reasonably be considered the most important, carbon might well come out on top.
Italian writer and chemist Primo Levi concluded his autobiographic novel The Periodic Table with a chapter dedicated to the element, in which he declared carbon, “the key element of living substance.”
There are some 43.5 trillion tonnes of carbon in the earth’s land, air and oceans, with billions upon billions more through the planet’s mantle and all through its solid core. Carbon also bonds readily to make news compounds. Scientists estimate that carbon is the keystone for 95% of known compounds.
With so much of the physical world made up of carbon, it has long been known to humans in stones, minerals, trees and plant life. It’s provided humans with building materials, diamonds and graphite, but what has been truly transformational in our relationship with carbon is when we burn it – or, in slightly more technical terms, combustion.
Carbon is humans’ oldest and, even today, most prominent source of energy. It was the fuel in the wood fires that first allowed humans to cook food and provide the energy needed for our bigger brains, and in the kerosene that launched the Apollo Mission rockets to the moon.
But it’s the CO2 produced from these reactions that has created the need for rapid decarbonisation today. And it was CO2 that provided the key for scientists to understand carbon itself.
It was the fuel in the wood fires that first allowed humans to cook food and provide the energy needed for our bigger brains, and in the kerosene that launched the Apollo Mission rockets to the moon.
Figuring out CO2
Joseph Black was a Boudreaux-born, Scottish physician and chemist who became professor of medicine and chemistry at Edinburgh University in 1766. Black taught there for 30 years, helping establish it as a major city in the scientific enlightenment of the 18th century.
But it was in 1754, while he was still researching his graduation thesis, that Black observed what he called ‘fixed air’. We know it today as carbon dioxide, or CO2.
While heating up some calcium carbonate Black recorded it producing a gas that was denser than air and could not sustain a flame or animal life. He subsequently demonstrated that this same ‘fixed air’ was produced by animal respiration and microbial fermentation. It was through CO2 that French scientist Antoine Lavoisier was able to formally identify carbon.
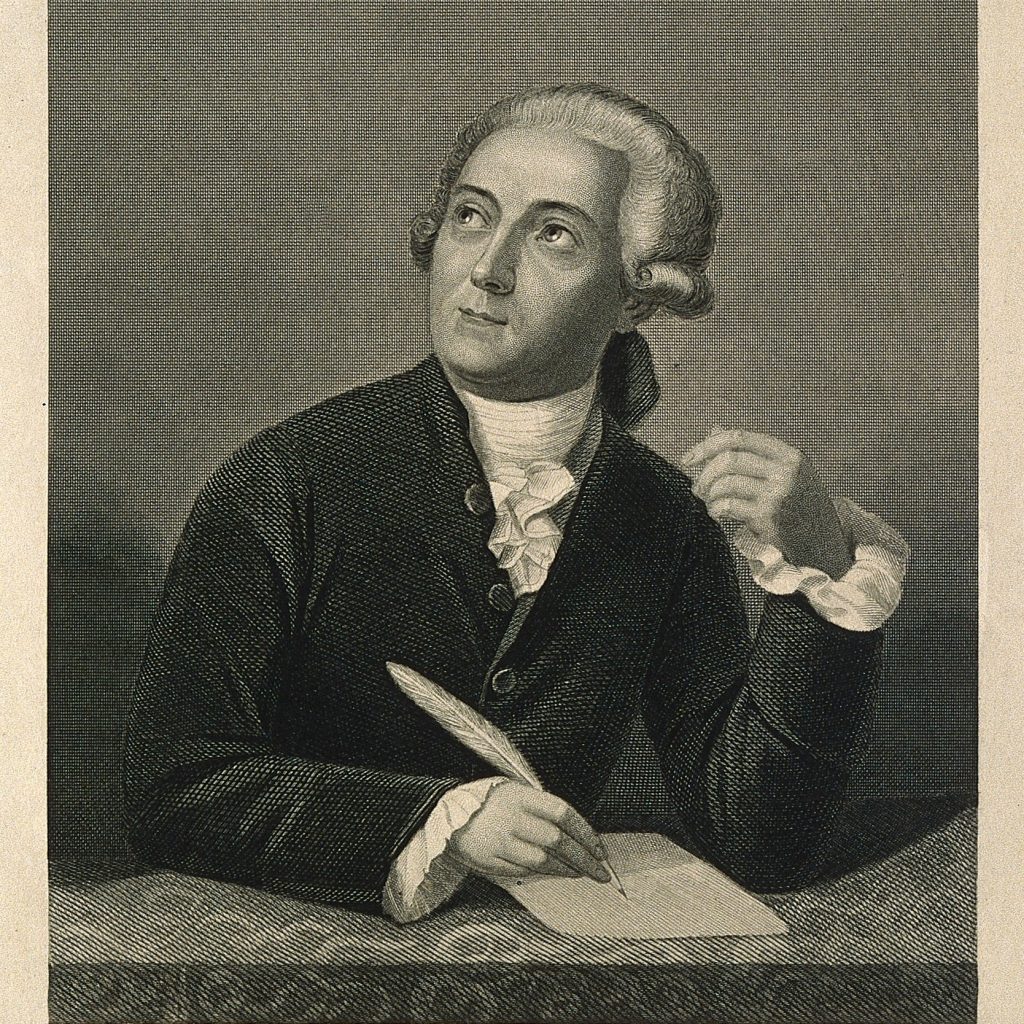
Antoine Laurent Lavoisier. Stipple engraving by W. C. Sharpe after J. L. David. Credit: Wellcome Collection
A fan of Black’s, Lavoisier had once written to the Scotsman declaring himself a ‘zealous admirer’ of his genius and of the ‘important revolutions’ his discoveries had caused in the sciences. Lavoisier would prove to be a revolutionary chemist too. In his career he formally named the elements carbon, hydrogen and oxygen, as well as uncovering oxygen’s role in combustion and respiration, before being guillotined in France’s Reign of Terror.
Where chemists today might use a Bunsen burner, Lavoisier deployed a giant magnifying lens to focus the sun’s rays and carry out his experiments in combustion. In 1772, he and some other chemists pulled together the cash to buy a diamond and promptly placed it in a glass jar to set alight under the magnifying glass’ intense rays.
When the diamond burned up completely, Lavoisier noted the overall weight of the jar was unchanged. The carbon in the diamond had bonded with oxygen in the jar to form CO2. To Lavoisier the experiment told him that both diamond, charcoal and ‘fixed air’ contained this same element, which he named carbon from the Latin ‘carbo’, meaning charcoal.
These Enlightenment era discoveries in combustion helped us understand what CO2 is and where it comes from, but it would not be until the combustion of fossil fuels at scale that the true effects of CO2 became clear.
Burning carbon, creating CO2
Fossil fuels, such as coal, natural gas and oil, are so called because they are made up of what was once organic, living materials. When these plants and animals die they decompose. Over millennia they are buried beneath layers of sediment and rock, and exposed to intense heat and pressures. The resulting materials are dense with the carbon that once made up the living matter. The carbon atoms are bonded together in long chains along with some hydrogen to make them hydrocarbons.
Hydrocarbons are very stable molecules, meaning they don’t react with surrounding substances. They also have a very high energy density, making them a very efficient fuel source. But when adding oxygen to the reaction, through combustion, the gaseous form of carbon, CO2, is released.
“When we burn a fuel that contains carbon, we can provide enough oxygen to get what we call complete combustion – that means the fuel releases as much energy as possible,” explains Dr Hannah Chalmers, from the University of Edinburgh. “The carbon and the oxygen like to bond together, and the most stable arrangement for them to be in is a carbon dioxide molecule.”
But CO2 is not just created by burning fossil fuels – it’s also a naturally occurring gas.
In the greenhouse
When humans – and every other animal on the planet – exhale, CO2 is a result of the chemical reaction that takes place in our lungs. It’s a part of the natural cycle of carbon that goes on around us.
CO2 naturally exists in the earth’s atmosphere having been released by respiration from animals, as well as microbes breaking down organic matter. It is also naturally absorbed from the atmosphere by different types of environments, from oceans to mangroves to forests, primarily by plants, to make glucose through photosynthesis.
The carbon-containing plants might then be eaten by animals which exhale the carbon as CO2. Other plants eventually die and decompose, with some of the CO2 released as they are broken down by microbes. Eventually, what’s left of the plant is buried underground and, millions of years later, the carbon is turned into materials like fossil fuels.
This movement of carbon around Earth is known as the carbon cycle. It keeps the levels of CO2 in the atmosphere balanced, where, along with numerous other gases, it plays the important role of regulating the earth’s temperature.
Solar energy from the sun travels to Earth where it is absorbed and radiated back out into space in the form of heat. But a group of gases, CO2 among them, prevent this heat leaving the earth’s atmosphere. This is known as the Greenhouse Effect and it’s by no means a bad thing. It’s because of this effect that the earth is warm enough to support life.
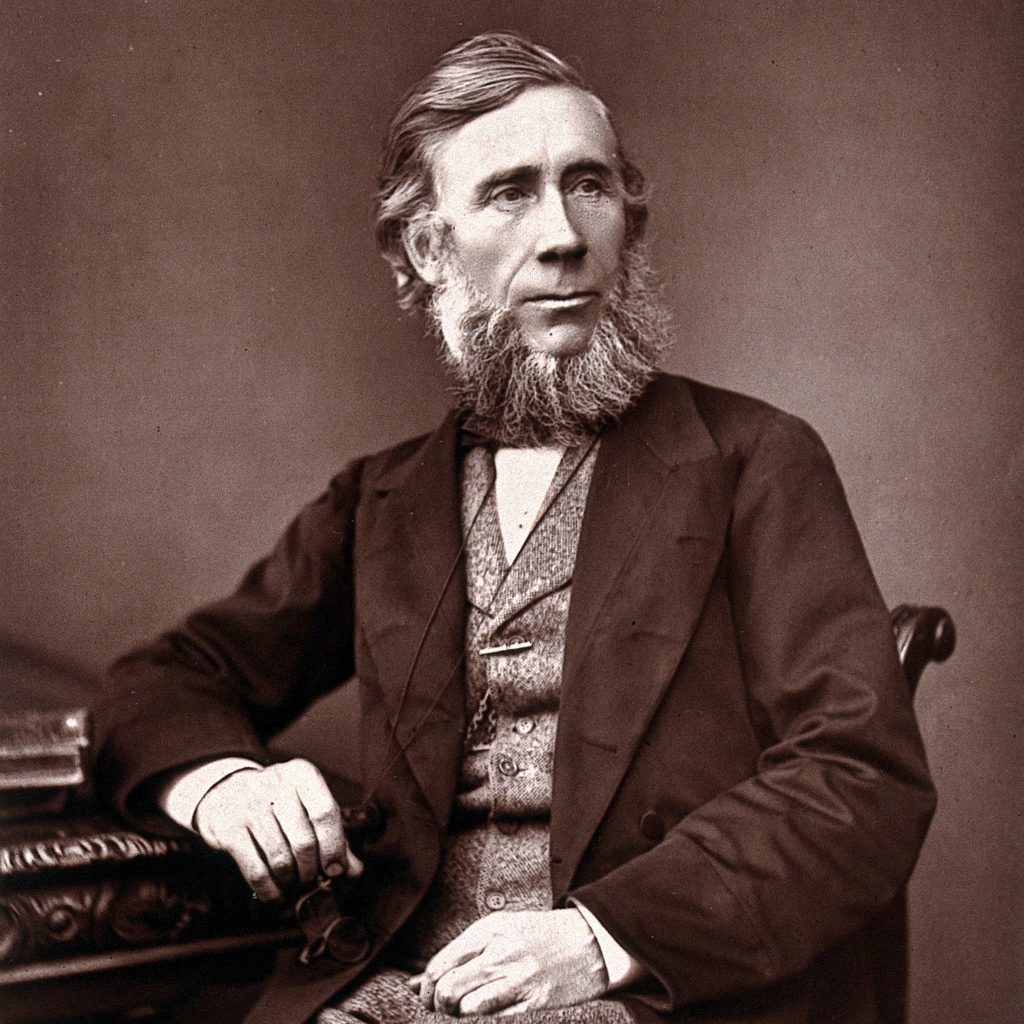
John Tyndall. Photograph by Lock & Whitfield. Credit: Wellcome Collection
This phenomenon was first noted by physicist John Tyndall in 1861, who concluded that this layer of gases “is a blanket more necessary to the vegetable life of England than clothing is to man”.
This necessary blanket is evident when comparing Earth to our neighbour Mars, where an atmosphere 100-times thinner than Earth’s means that its warmest spots still drop to minus 100 degrees Celsius at night – even in summer.
However, while Greenhouse Gases help make our planet liveable, excessive levels trap too much heat between the earth and its atmosphere. The result is soaring temperatures on the earth’s surface, ultimately making it less habitable.
The gases that cause this effect make up less than 0.03% of the earth’s atmosphere, but they can have a huge impact. Methane, nitrous oxide, ozone and even water vapour are all Greenhouse Gases, but it’s CO2 that is particularly damaging and prevalent in the atmosphere.
Greenhouse Gases are naturally removed from the atmosphere. Water vapour only spends about nine days in the atmosphere before it condenses into clouds and falls as rain as part of the water cycle. Methane is removed naturally from the atmosphere in about 12 years, through chemical reactions, while nitrous oxide takes around 114 years.
CO2, though, can last for much longer once it’s in the atmosphere, lingering for 300 to 1,000 years. This means CO2 emissions transcend generations and human lifetimes, compounding and becoming a bigger and bigger part of the atmosphere, exacerbating the Greenhouse Effect.
Slow progress to tackling emissions
The effect of CO2 on global temperatures has been recognised since as long ago as 1896, when Swedish chemist Svante Arrhenius concluded that burning coal would enhance the natural greenhouse effect. Like Tyndall before him, Arrhenius though the idea of a warmer Earth was entirely positive.
The correlation between CO2 concentrations and temperature rises were further confirmed in 1938, when British engineer Guy Callendar combined records from 147 weather stations around the world. He believed this increase in CO2 would be beneficial in staving off another ice age. What became known as the Callander effect was dismissed by meteorologists at the time.
It was not until 1965 that US oceanographer, Roger Revelle warned president Lyndon Johnson about the danger of rising sea levels, melting ice caps and freshwater acidification linked to fossil fuel emissions. A decade later Wallace Broecker put the phrase ‘global warming’ into the public lexicon with his article ‘Climatic Change: Are We on the Brink of a Pronounced Global Warming?’
International coordination did not come until the 1980s, with the Kyoto and Montreal protocols, designed to tackle Greenhouse Gases and chemical damage to the Ozone layer, respectively.
It’s now clear that human activity is causing the CO2 levels to rise at an alarming rate. Currently, the concentration of CO2 in the earth’s atmosphere is around 412 parts per million (ppm), up as much as 47% from the beginning of the Industrial Age around the 1760s, and 11% from 2000.
The combustion of fossil fuels is taking carbon that had dropped out of the natural carbon cycle millions of years ago and flooding it back into the atmosphere as CO2. While we now know how to cut CO2 emissions, we are also aware of how big the challenge that lies ahead is.
Chapter 3:
Capturing carbon
An often cited and debated anecdote about former UK Prime Minister Margret Thatcher, is that before entering politics she worked at food conglomerate J. Lyons & Company. While there, legend claims, she helped invent soft scoop ice cream.
Her degree in chemistry may have led to the job of formulating frozen treats, and it also came into play in a 1989 speech she gave to the UN, where she said: “We are seeing a vast increase in the amount of carbon dioxide reaching the atmosphere … At the same time as this is happening, we are seeing the destruction on a vast scale of tropical forests which are uniquely able to remove carbon dioxide from the air.”
It would still take 26 years of action and further research before the Paris Agreement was signed in 2015. However, while policy change came slowly, the technology and thinking around how to reduce CO2 emissions and remove it from the atmosphere have both evolved.
Overall, across the whole UK economy, 351.5 million tonnes of CO2 (MtCO2) were emitted in 2019, according to National Statistics, a 60% reduction from 1990 levels. The growth of renewables, from 5.2 gigawatts (GW) of capacity in 2010 to 38.5 GW in 2019, has been a huge driver of this, with emissions from power sources 64% lower in 2019 than in 2010.
However, even with this progress it’s forecast that some emissions will remain in the UK economy. To reach net zero by 2050 will require CCUS. But how exactly is carbon captured?
Capturing Carbon
Capturing carbon, transporting it and storing it thousands of metres underground sounds like it could be the work of science fiction. In reality the technology is well understood, proven and surprisingly straightforward.
In a power station, for example, a fuel, such as coal is burned to generate steam and power a turbine. The reaction that takes place in the boiler can produce a range of chemicals including sulphates, nitrous oxides (NOx) and, of course, CO2. There have long been efforts to try and clean up so-called flue gas with technologies, such as boosted-over-fire-air (BOFA) to reduce NOx and desulphurisation equipment. However, the massive amount of CO2 produced from fossil fuels is more difficult to deal with. This is where CCUS steps in.
Flue gas is fed into a piece of equipment that is often known as an absorption tower or column. Here, a solvent attaches to the CO2, pulling it out of the flue gas and down the tower, leaving the CO2-depleted flue gas to exit. The CO2 is then taken away to be used or sequestered permanently below the ground.
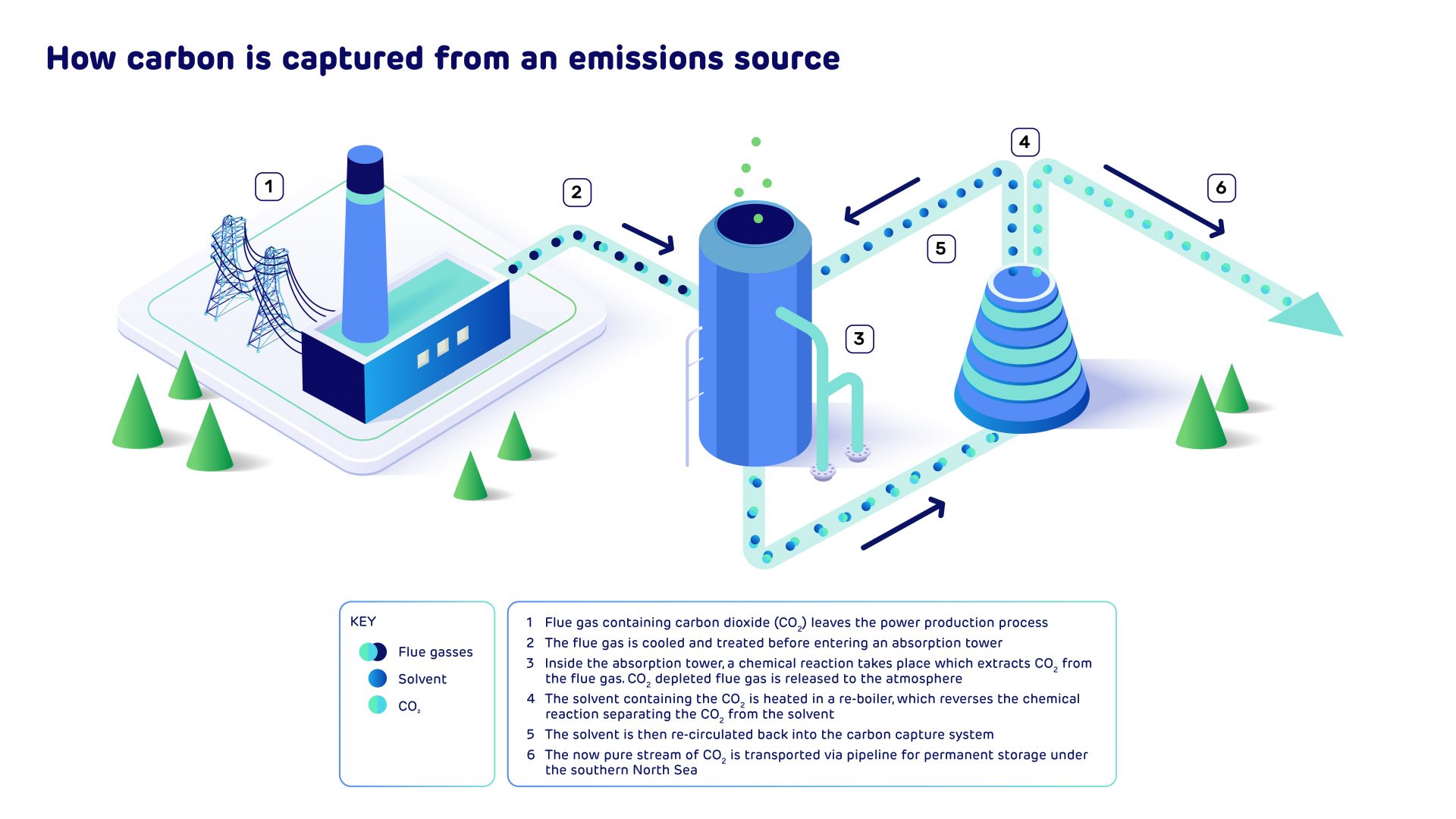
The process is simple, but the trick is in the science behind the solvent that can capture the CO2. Lots of companies have their own top-secret take on what goes into a solvent that is capable of delivering CCUS at scale, but generally they are made of amines.
Amines are organic compounds made up of one or more nitrogen atoms connected to two hydrogen atoms and a group of other atoms. What’s really useful about amines is not just that they readily bond with CO2, but that the reaction can be reversed by applying heat.
This means that the same amines can be used in a cycle: capturing CO2 in the absorption tower, dragging it down to a reboiler where they are heated up and separated. The amines can then re-enter the absorption tower to get back to work grabbing more CO2, which is transported away in a separate stream to be used, stored or permanently sequestered. But it isn’t only after a reaction takes place that CO2 can be captured.
Pre-combustion capture – taking the CO2 out before it can escape
Rather than being captured and stored after a fuel has been combusted, CO2 can instead be taken out of a fuel beforehand, in what is known as pre-combustion capture. The most common way for this to occur is for a fuel, such as coal or even biomass, to be converted from a solid into a gas by applying heat and pressure – but preventing it from combusting.
The synthesis gas, or syngas, that is produced is a mixture of hydrogen, carbon monoxide, CO2, and small amounts of other gases. The CO2 can be removed from this gassy mixture using solvents (as in post-combustion CCUS), solid sorbents that absorb CO2, or a membrane that removes CO2 as the gas passes through it. The result is a stream of CO2-depleted gas that is less corrosive in pipelines and can also be combusted as a fuel source.
Because the concentration of CO2 in syngas is much higher (as much as 50% compared to ~15% in flue gas from a coal power station) it is actually easier to remove. However, the energy needed to produce the syngas makes it less efficient than post-combustion capture.
But it’s not just in power generation where CCUS will be vital. According to the Committee on Climate Change’s 2020 Progress Report Climate Change Committee’s 2020 Progress Report, industry, which includes the use of fossil fuels as well as carbon-intensive processes such as steel and cement manufacturing, contributed 21% of the UK’s Greenhouse Gas emissions in 2019. These vital sectors will also require CCUS to reach net zero.
From carbon capture to carbon negative
CCUS is not a silver bullet that can be applied to every essential part of the economy. Road transport accounted for 24% of the UK’s emissions in 2019. While there is rapid development in electric vehicles and a proposed ban on new sales of petrol or diesel cars coming into effect in 2030, eliminating every fossil-fueled vehicle from the UK’s roads by 2050 is a tall order.
Agriculture and aviation, which accounted for 9% and 8% respectively, of the UK’s 2019 emissions are other vital sectors that will be massively difficult, or potentially impossible, to completely remove CO2 emissions from. These emissions that are forecast to remain in the economy, even with extensive decarbonisation, are known as residual emissions.
It’s difficult to predict just how much CO2 will still be emitted over the coming years. Events like the COVID-19 pandemic can cause unexpected shifts. The National Grid’s 2020 Future Energy Scenarios (FES) Report, lays out a Steady Progress scenario in which decarbonisation is slow and limited to power and transport sectors. In this forecast there is still 258 MtCO2e being emitted in 2050.
It’s because of this that it is essential the UK deploys not just CCUS but negative emissions that can reduce the overall amount of CO2 in the atmosphere.
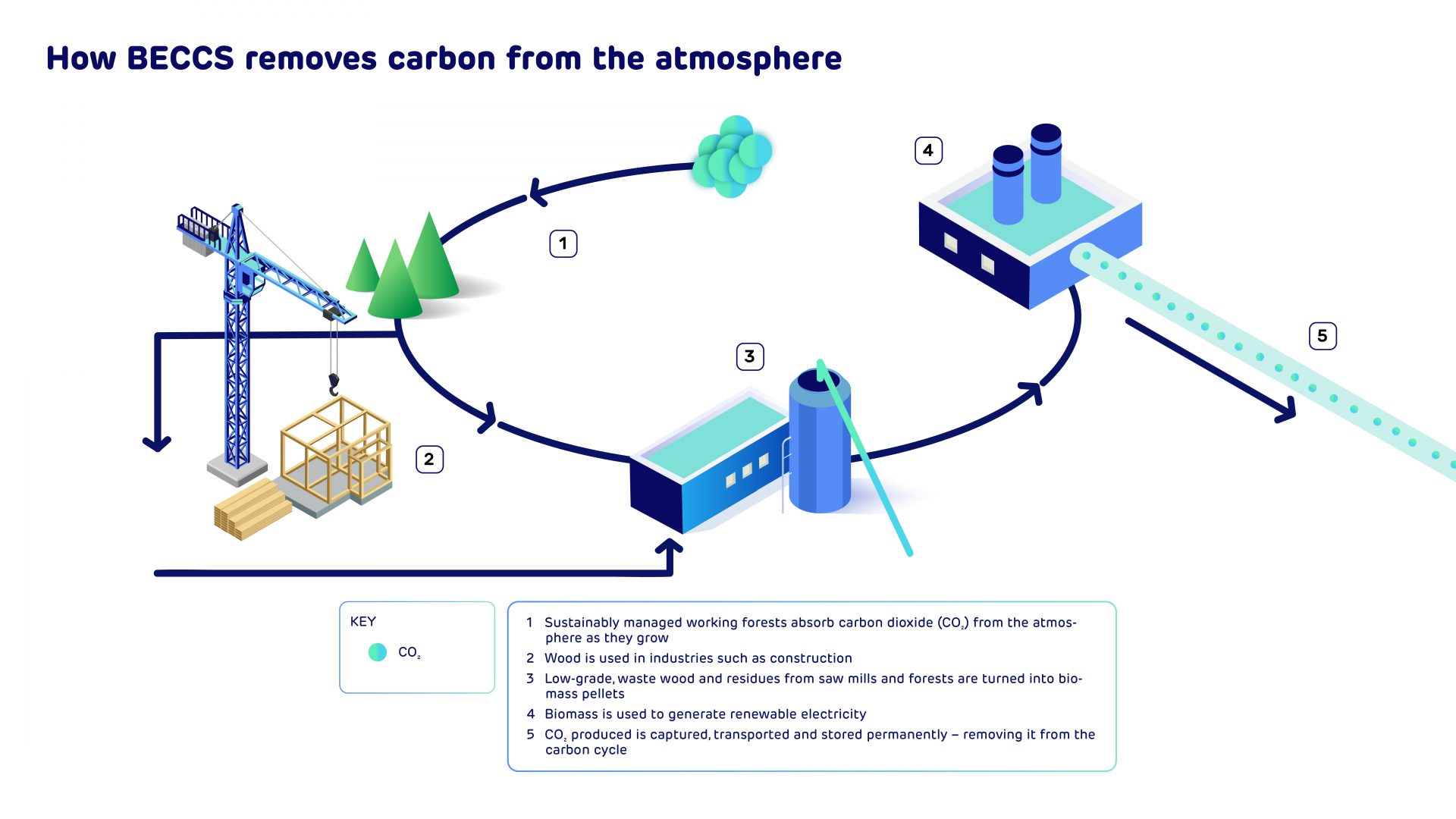
Find out more about Bioenergy with carbon capture and storage (BECCS) and negative emissions here
There are many ways to approach negative emissions, such as reforestation and afforestation or making ocean vegetation more effective in its CO2 absorption. There is also direct air carbon capture and storage (DACCS). This more nascent technology operates in a similar way to CCUS using methods such as amines, sorbents or membranes to capture CO2 from ambient air anywhere in the world.
Where DACCS becomes trickier than conventional CCUS, is the low density of CO2 in ambient air. As it makes up just 0.04% of the air around us, the amines or solvents used in DACCS are like needles in a haystack compared to those in a richer CO2 stream from bioenergy or other processes.
Bioenergy with carbon capture and storage (BECCS), is uniquely placed. BECCS’s ability to remove CO2 from the atmosphere and store it permanently underground, while generating renewable electricity, means it is now considered vital to reaching net zero.
The importance of BECCS is emphasised more strongly than ever in the FES report, which estimates that BECCS in the UK has the potential to generate 62 million tonnes of negative carbon emissions annually by 2050. It’s equal to a quarter of the 258 MtCO2 that may remain in the worst-case scenario, and vital to bringing the country to net zero as the clock ticks down to 2050.
Laying out the numbers to getting carbon under control is one thing; building and operating CCUS, BECCS and DACCS at the scale needed to hit those targets is another. All three technologies are scientifically proven. The challenge is in finding the economic model that can cover the upfront cost of developing and building the infrastructure needed deploy them at scale.
Chapter 4:
Finding a model in an emergency
On the northern bank of the River Forth, across from Falkirk in Scotland, lies the remains of what was once Europe’s biggest coal power station. When it opened in 1973, Longannet Power Station could generate 2.4 GW of electricity, with its four boilers blasting through 4.5 million tonnes of coal each year.
With the demolition of much of the station’s turbine hall and coal facilities following its closure in 2016, little remains beside debris. It’s a symbol of the fossil fuel past that the UK is rapidly leaving behind. In 2021 the 183-metre-tall chimney that has dominated the Forth’s skyline is scheduled to come down too.
But Longannet could also be seen as a missed opportunity for the UK’s first large-scale carbon CCUS facility.
In 2009 the power station was the last competitor standing in a Government contest to award £1 billion to develop the UK’s first commercial-scale carbon capture project. Having successfully demonstrated a 1 megawatt (MW) project, which captured over 90% of CO2 emissions, the power station planned to partner with Shell to use existing gas facilities to transport the CO2 to the North Sea and store it in depleted gas fields via the oil firm’s Goldeneye Gas platform.
However, in 2011 the plan and funding were scrapped, and with it Longannet’s fate for demolition was sealed. The reason: there was no economically viable way to develop and construct the infrastructure needed to deploy CCUS at a large scale.
It’s a problem that has plagued CCUS development to date. But new approaches and a new governmental need to achieve net zero by 2050 mean the UK is finally on the verge of implementing the technology at the scale needed.
Attempts to deploy CCUS at scale
There are already examples of CCUS technologies being deployed at scale around the world. Petra Nova power station in Texas got further than Longannett, deploying a 240 MW CCUS project on one of its coal-generating units, with the captured CO2 sold for enhanced oil recovery.
It makes economic sense; oil companies want to acquire the CO2 to extract more oil from wells, while the power stations are financially rewarded for decarbonising. However, when oil prices fall it damages the market for enhanced oil recovery too, as a result Petra Nova failed to find an economically sustainable way to deploy CCUS at scale.
But moreover, if the world is to drastically cut its CO2 emission to the levels necessary to prevent climate change, then it needs to move away from all fossil fuels.
As the importance of CCUS and negative emissions become increasingly apparent in decarbonisation, government support and policy will be crucial in delivering rapid progress.
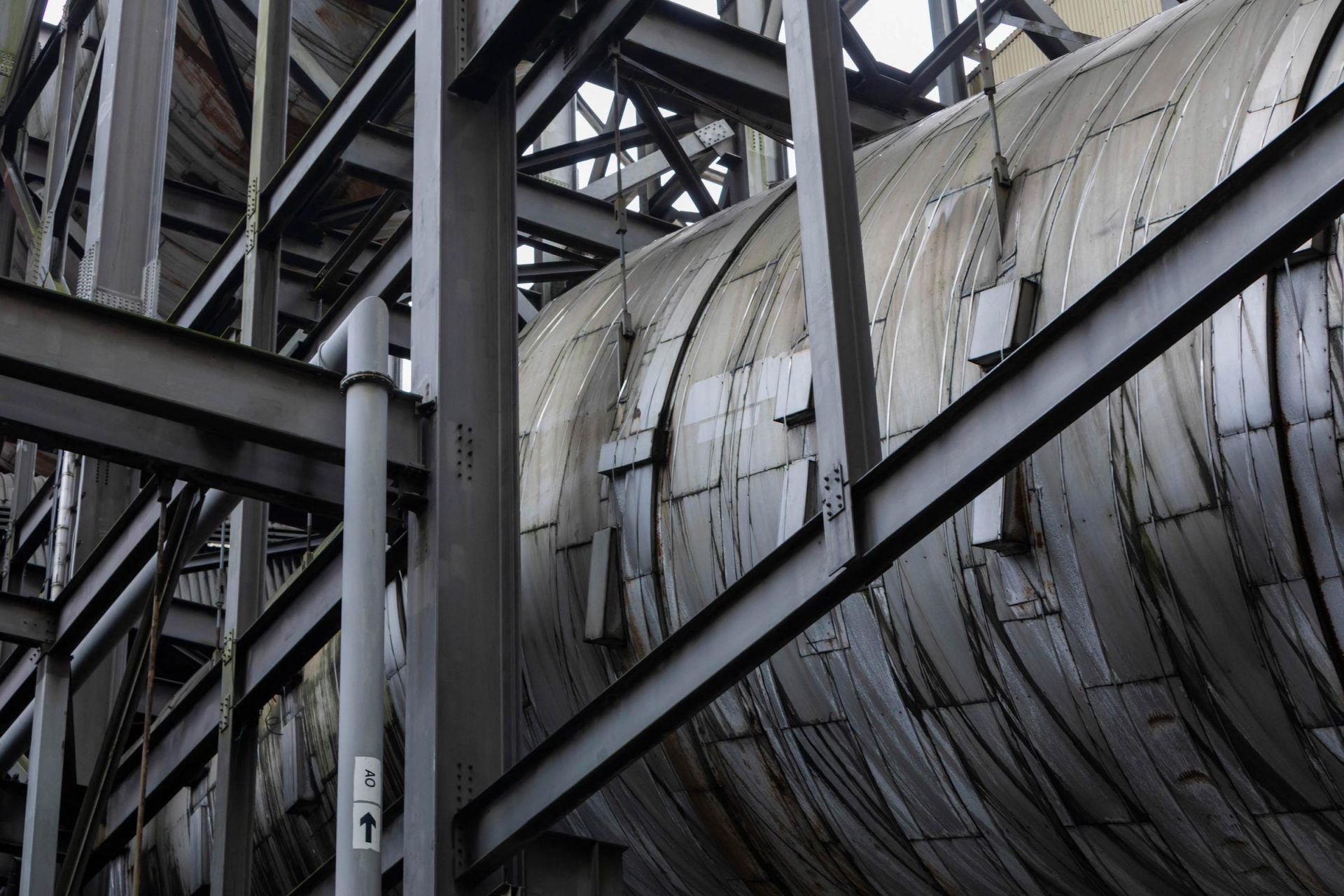
The White Rose Project was among a second wave of attempts to develop commercial CCUS in the UK. The plan aimed to capture CO2 at a new coal-fired power station on Drax’s site near Selby in North Yorkshire and transport it to storage sites in the North Sea. But it was stopped when the Government cut short another CCUS commercialisation programme in 2015.
In the five years since, however, the landscape around decarbonisation and the thinking around deploying CCUS at scale has changed. The UK’s legal commitment to reaching net zero by 2050 means there is a greater awareness and pressure on Westminster to implement CCUS as a rapid tool for decarbonisation.
“Today there is a very different political atmosphere than the past,” says Chris Gent, Policy Manager at the Carbon Capture and Store Association. “The UK’s statutory net zero target means that all sectors are now under the magnifying glass to push towards carbon neutrality.”
The thinking around how to share both the costs and benefits of a large scale CCUS system has also evolved. In the past, projects centred around one source of emissions, such as a single power station, which would capture and transport CO2 to be stored, bearing all the cost of doing so.
“Today there is a very different political atmosphere than the past. The UK’s statutory net zero target means that all sectors are now under the magnifying glass to push towards carbon neutrality.”
Chris Gent, Policy Manager at the CCSA (Carbon Capture & Storage Association)
Now, however, there is an emphasis on industrial clusters of CO2-intensive factories, manufacturing plants and power stations that can all capture and divert their CO2 into shared pipes that transport it to storage sites and deliver significantly larger reduction in overall emissions. “The cluster approach offers significant economies of scale” says Gent “and enables multiple emitters that share common CO2 infrastructure, to achieve net zero.”
Clusters depend on cooperation and collaboration across different industries and even geographies of the UK. In March 2020 the government announced it would commit at least £800m to support the development of CCUS infrastructure in two industrial clusters. Prime Minister Boris Johnson raised this commitment to £1bn just eight months later.
Sustained government thinking beyond just five-year election cycles is required to put in place the schemes needed to bring parties to the table and drive the investment needed to bring projects to fruition.
The policies needed to make CCUS a reality
One of the biggest hurdles for CCUS is the investability of the technology. But this is not unusual in energy. Wind and solar power generation were once considered uneconomic and too expensive to ever really be deployed at scale. However, thanks in no small part to sustained support and revenue stabilisation schemes, they now make up a significant part of the UK’s energy mix, with wind accounting for 21% and solar 9% of 2020’s second quarter electricity generation.
There are a variety of ways to approach subsidies to get projects off the ground. Existing mechanisms could also be applied or tailored to CCUS to ensure it is a viable investment.
One device that could be used to develop a market for CCUS are contracts for difference (CfDs). These contracts are currently used in low-carbon generation space, between electricity generators and the government-owned Low Carbon Contracts Company (LCCC). However, more than just CCUS deployment, policies must incentivise the negative emissions technologies deemed essential for the country to reach net zero in 2050.
Contracts for difference
Supporting investments and protecting consumers
- The Low Carbon Contracts Company (LCCC) pays power generators the difference between the cost of generating low-carbon electricity (known as a strike price) and the price of electricity in Great Britain’s wholesale power market.
- If the costs of generating a megawatt of electricity (with the cost of CCUS infrastructure added on) is greater than the price the electricity is being sold, the LCCC will pay the generator the difference to prevent them making a loss.
- Conversely, if the power price in the market is higher than the strike price generators pay the difference back to the LCCC.
- Consumers are protected from price spikes and generators are protected from market volatility or big drops in the wholesale price of power, offering the security to invest in new technology.
- CfDs last many years, meaning they transcend political cycles and the costs per megawatt can be reduced with longer contracts.
Mechanisms like CfDs would operate under different models and conditions for different types of CCUS installations, including power generation with CCUS, CCUS on industrial emitters and BECCS. The effect could be that the strike price acts as a continual subsidy to CCUS projects on top of the wholesale price of power.
Supporting negative emissions through taxes
Carbon tax
- Current systems, such as the UK Carbon Price Floor, require emitters to pay £18.08 per tonne of CO2.
- These existing taxes from companies that contribute to emissions could be used to fund technology reversing the process.
Tradable tax credits
- Other systems such as the EU Emissions Trading System (ETS) set a limit on company emissions and require them to buy or trade allowances, with fines for exceeding limits.
- These fines could be used to fund negative emissions projects.
- Other approaches could require fossil fuel suppliers to offset the emissions of their products by purchasing certificates awarded to negative emissions providers.
- This would inevitably force fossil fuel companies to raise their prices, making them less attractive as a source of energy and sharing the costs further down the supply chain.
A shortcoming of tax-based systems is their susceptibility to changes in governments, but it offers a significantly cheaper route than direct grants or long-term contracts that transcend changes of government.
Different policies and systems can also work in conjunction, where contracts such as CfDs provide foundations for CCUS and BECCS operations, which are rewarded for through carbon pricing.
The challenge of finding a perfect policy to launch CCUS and BECCS at scale in the UK is also the perceived complexity of the process.
“CCUS is not a simple technology to explain to the public compared to renewable energy,” says Gent. “This is why it is so important that we get CCUS projects in operation, so that the public can see that the technology works and delivers benefits for local communities.”
While the technologies needed to make CCUS and BECCS are proven and tested at scale, there’s still a story to be told about where all that CO2 goes.
Chapter 5:
Locking carbon away
The North Sea, off the UK’s Eastern shoulder, is closely connected to the country’s past. From migrating Angles and Saxons to Viking raiders and German U-Boats, events in the North Sea have shaped the country. What lies below the seabed has also had an important influence on the modern UK and could play an even bigger role in its future.
Kilometres below the icy waves and seabed lie abundant sources of oil and natural gas which have served to power Great Britain and its maritime neighbours in recent decades. However, as countries shift away from CO2-emitting fossil fuels, the layers of geological formations below the North Sea offer a suitable site to store captured CO2 safety and permanently.
Just as the technology of capturing CO2 from emissions is already proven, the practise of injecting it into geological formations in the earth’s crust is decades old across the oil industry. However, the function of injecting CO2 into Permian Basin oil wells is very different to permanently storing it and preventing it entering the atmosphere.
“When recovering more oil by pumping in CO2, the aim is to displace the oil towards your production wells. When pumping CO2 into the ground for sequestration, the idea is to keep it there,” says Professor Andy Woods, Head of the BP Institute at Cambridge University. “There is a lot of knowledge from the oil industry that’s helping to inform CCUS, but the objectives are different.”
There is still much to learn about how CO2 spreads through different rock formations and how to store it as efficiently as possible, and research is well underway. But before CO2 can be locked up underground, it has to be transported.
The pipeline to storing emissions
Gas is transported around the world in trucks or ships, but the most efficient way is by pipeline. The natural gas used around the UK is already piped in from across the North Sea. The 1,166km Langeled pipeline from the Norwegian Ormen Lange gas field was the longest undersea pipeline in the world when it began operating in 2007.
The UK also has significant experience and regulation in place to move natural gas around the country – piping CO2 doesn’t take much of a leap in knowledge. However, there are some other ways to ensure CO2 gets around as efficiently as possible.
“CO2 is transported in a special physical state where it has some properties that are similar to liquids and others that are like gases. It has the density of a liquid, but the viscosity of a gas,” explains Chalmers. “It means it can travel down a pipeline in quite large quantities but behaves differently to say, natural gas.”
Ultimately though, CO2 is a very stable molecule and even in the unlikely event of a pipeline issue, it would not explode as natural gas might. Where to transport the CO2 to, however, is a matter of finding the right locations.
Using geology to lock up emissions
There are a number of reasons why geological features below the ground make sense as CO2 storage sites. For one, there is a massive amount of available space to store CO2 in the layers of rock that lie through the earth’s crusts. Leaving huge amounts of CO2 in man-made tanks above the ground would take up large amounts of space and require continual costs to maintain, while also leaving the waste more vulnerable to human errors or accidents.
“Oil and gas fields are good hosts because the type of rock is known and there has already been geophysical mapping and understanding around these formations.”
Professor Andy Woods, Head of the BP Institute at Cambridge University
Another reason geological formations make sense is because they are already well understood – a result of more than a century of research into the kilometres of rock layered beneath the earth’s surface, largely by the oil and gas industry. It’s somewhat ironic that now depleted fossil fuel sites could hold the key to negative emissions and a carbon neutral future.
“Oil and gas fields are good possible hosts because the type of rock is already known and there has already been quite a lot of geophysical mapping and understanding around the structure of these formations,” says Woods. What’s key is finding porous, permeable formations that can allow fluids, such as CO2, to pass through them.
A porous rock, such as sandstone, is composed of a series of sand grains that have been pressed together. They are in contact with each other, but there are gaps, or pores, between the grains. The permeability of rock describes how easy it is for a fluid, like CO2, to move between the pores. Depending on how deep underground the rock is and how much pressure is being applied on the grains, the size of pores will differ. However, they remain very small – between 100 micrometres (1 millionth of a metre) and up to about 1 millimetre.
It’s in these pores, that oil and natural gas are found, often in close proximity to each other. Once oil and natural gas are removed these pores offer a permanent storage space for CO2.
Another possible target for storing CO2 are saline aquifers. These are porous layers in the geologic strata, filled with brine or saline water. When CO2 is injected into the rock formation it floods into the pores, displacing the brine or remnants of oil and gas and spreading out laterally across the topology, eventually making its way into structural traps in the anticlines.
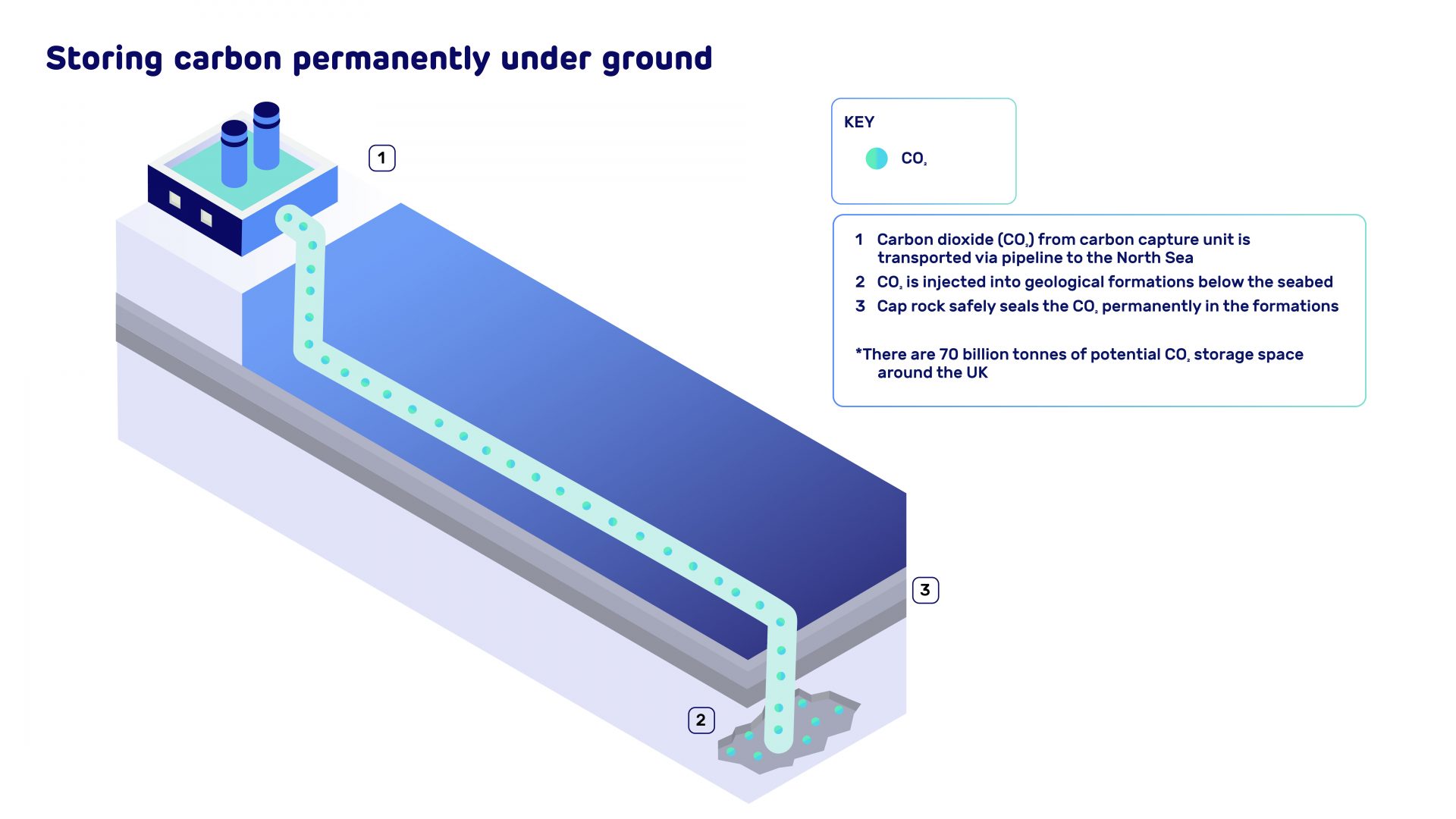
Another geological feature needed at a CO2 storage site is a cap rock or seal rock. This is a layer of non-permeable rock that sits above the formation into which the CO2 is injected. The cap prevents the gas from continuing its natural journey upwards, sealing it into anticlines for the long term.
“Some of the CO2 will remain as CO2 in pore spaces, some of it will be dissolved in the water, and some of it may react with other elements to form carbonate minerals,” explains Woods. “Ultimately, once it’s turned into carbonate minerals, that’s geological sequestration, because it’s turned into part of the rock.”
One study, published in the journal Nature, suggests more than 98% of injected CO2 will remain stored for over 10,000 years. But besides locking it away, there is also the potential to make use of captured carbon.
The paradox of using carbon
In many of its forms, carbon is a highly valuable element and CO2 has its uses too, the carbonated drinks industry being one. Today, 88% of CO2 usage around the world is in enhanced oil recovery, promoting fossil fuel extraction. However, carbon’s ability to form very stable bonds with other molecules, means it can be used in other ways, such as providing an alternative to materials like plastics and concrete, as well as in fuels. But there are limitations to using CO2.
“Because CO2 is so stable, it can be difficult to make products from,” explains Chalmers. “It requires a lot of energy to break the carbon and oxygen atoms apart so they can be used in other molecules. That energy has to come from somewhere, so the overall process might be difficult to implement.”
There is also the matter of ensuring that CO2-created products are compatible with a net zero future. For example, demand for captured CO2 should not encourage a power station to burn fossil fuels to profit from the CO2 produced. What’s more, the products created must lock-in the carbon for the long term. Single use plastics that end up in landfills or incinerators only delay, rather than prevent the CO2 entering the atmosphere. Similarly, fuels can only be classed as carbon neutral if the CO2 they are made from is captured through a carbon neutral or carbon negative process.
Products created from captured carbon dioxide must lock-in the carbon for the long term.
The market for utilising CO2 is still in its infancy, and in the immediate future it is unlikely to be a major destination for captured emissions. “Carbon usage may only make up a fraction of the amount of carbon captured in the future,” suggests Gent. “There’s lots of innovation and potential applications but it will be a relatively small volume compared to storage.”
The question, then, is how much storage space is available in which to lock CO2 away permanently?
How much space is there to store carbon?
The International Energy Agency (IEA) estimates that an accumulative 100 billion tonnes (or 100 gigatonnes) of CO2 must be stored by 2060 to limit global warming to a two degree Celsius increase – in a scenario in which one-sixth of global emissions reductions come from CCUS. It sounds like a lot, but it’s well within the potential storage capacity of many regions.
According to the Global CCS Institute, Europe alone has more than 300 gigatonnes of available geological storage space – the US is home to the known geology to store an estimated 10 trillion tonnes of CO2. Many countries possess over 1,000 tonnes of CO2 storage capacity per person, which is several times an average person’s lifetime carbon footprint.
The UK has an estimated 70 billion tonnes of potential carbon dioxide storage space.
Not only is storage capacity available, some of it is also already in use. Norwegian energy firm Equinor currently stores 1.5 million tonnes of CO2 a year in the North Sea through its Northern Lights project.
The North Sea, with its expansive layers of porous sandstone, also offers the UK an estimated 70 billion tonnes of potential CO2 storage space. It means that if negative emissions technologies (which actively remove emissions from the atmosphere) were to capture and store the equivalent amount of CO2 as the 258 million tonnes expected to remain in the UK economy in 2050, it would take up just 0.36% of the available storage space.
With deep knowledge, proven science and ample storage within reach, the UK is well-placed to make CCUS a key driving force in its journey to a net zero future.
Chapter 6:
CCUS in the net zero future
Look around the UK today and it’s difficult to find something that won’t need to change by 2050.
Buildings will be lit by electricity from renewable sources, heated by alternative fuels and optimised to be as energy efficient as possible. Cars will be powered by batteries, while heavy vehicles, ships and planes will use new fossil-free fuels. The materials that make up the world around us will also change. There will be greater use of wood and materials that lock in CO2 captured from the atmosphere, while steel and cement, two emissions-heavy materials, will be manufactured with CCUS in place.
The electricity generation industry, through a combination of increased renewables and negative emissions, could even reach net negative emissions as early as 2033. But while emissions will be reduced massively across many industries, some will inevitably remain.
Negative emissions techniques and technology will be needed in bringing overall levels to net zero. But beyond 2050, negative emissions offer even greater potential: to help reverse the climate changing impact of emissions of the past.
Building a diverse energy future
The CCC estimates that, with extensive decarbonisation measures in place, the UK can reduce its emissions by 95-96% from 1990 to 2050. Cutting the remaining 4-5% of CO2 emissions will require greater measures such as lower beef, lamb and dairy consumption, as well as greater emissions abatement, such as increasing forest cover to 17% by 2050. Other means will also be necessary, like enhancing the amount of CO2 absorbed by rock weathering or increasing the amount of carbon held in soil using biochar, a substance similar to charcoal that is created when biomass is combusted.
However, technological approaches will also be essential in removing residual emissions that remain in the atmosphere. These can be more rapidly deployed, and their effectiveness accurately measured to account for the amount of CO2 being removed.
BECCS, in particular, is essential in being both a source of emissions removal and a source of reliable electricity generation – one that can support an energy system dominated by intermittent renewables.
“Power plants with CCUS or BECCS can still start up or shut down at any time, which offers synergies with other low carbon technologies that are less able to turn on and off,” says Chalmers. “You need an energy system to have a mixture of different technologies. There are many great things about wind power, but there are times when it needs a friend. CCUS can provide a nice reliable low carbon friend to wind.”
BECCS, in particular, is essential in being both a source of emissions removal and electricity generation.
Hydrogen is also forecast to have a big impact on decarbonisation, acting as a zero-carbon emissions alternative to natural gas in heating and homes, or to petrol and diesel in large vehicles, and even as an alternate gas in power generation.
The key advantage of hydrogen is that, when combusted, it produces just water vapour and heat. But producing it from water depends on renewable or carbon-neutral electricity sources, while extracting it from natural gas would require CCUS.
The interplay between different technologies and industries is essential in bringing about economy-wide transformation that transcends traditional sectors. Shared CO2 transport and storage sites, and interdependent power and hydrogen generation, are key to constructing net zero industrial clusters. By decarbonising the areas of highest emissions, carbon savings can be extended to rest of the country and beyond.
Exporting net zero
The Humber is one of a number of regions around the UK where different companies and industries, from power to steel to chemicals, are partnering to form clusters and combining decarbonisation efforts. Zero Carbon Humber is a partnership of companies planning to decarbonise the most carbon-intensive industrial cluster in the country, an area that emits 12.4 MtCO2 a year.
BECCS will require new skills, new people, new mindsets and new thinking.
Carl Clayton, Head of BECCS at Drax
“From an engineering point of view this is a huge project,” says Carl Clayton, Head of BECCS at Drax. “It will require new skills, new people, new mindsets and new thinking. It’s a big shift – but as an engineer you enjoy it. It challenges you to work through problems collaboratively and draw upon a wide range of expertise.”
As well as being environmentally essential, large scale decarbonisation of the UK’s industrial clusters are also economically vital. In the Humber alone, 20% of economic value comes from energy and emissions-intensive industries, such as steel – as many as 360,000 jobs are supported by industries like refining, petrochemicals, manufacturing and power generation.
But beyond just leading a UK economic recovery, carbon capture and negative emissions offer a technology and knowledge base the UK can export to help the world decarbonise. A report by Vivid Economics for the Department of Business, Energy and Industrial Strategy found that opportunities to export CCUS could add £520 million a year to the economy and support 6,000 jobs by 2050.
However, CCUS and BECCS is not just about the interconnection of industries and energy, but of human activity and our world.
From fossil fuels to forests
In his concluding chapter on carbon Primo Levi highlighted plants’ ability to convert carbon into glucose through photosynthesis. He wrote if it “were not a common daily occurrence, on the scale of billions of tonnes a week, wherever the green of a leaf appears, it would by full right deserve to be called a miracle.”
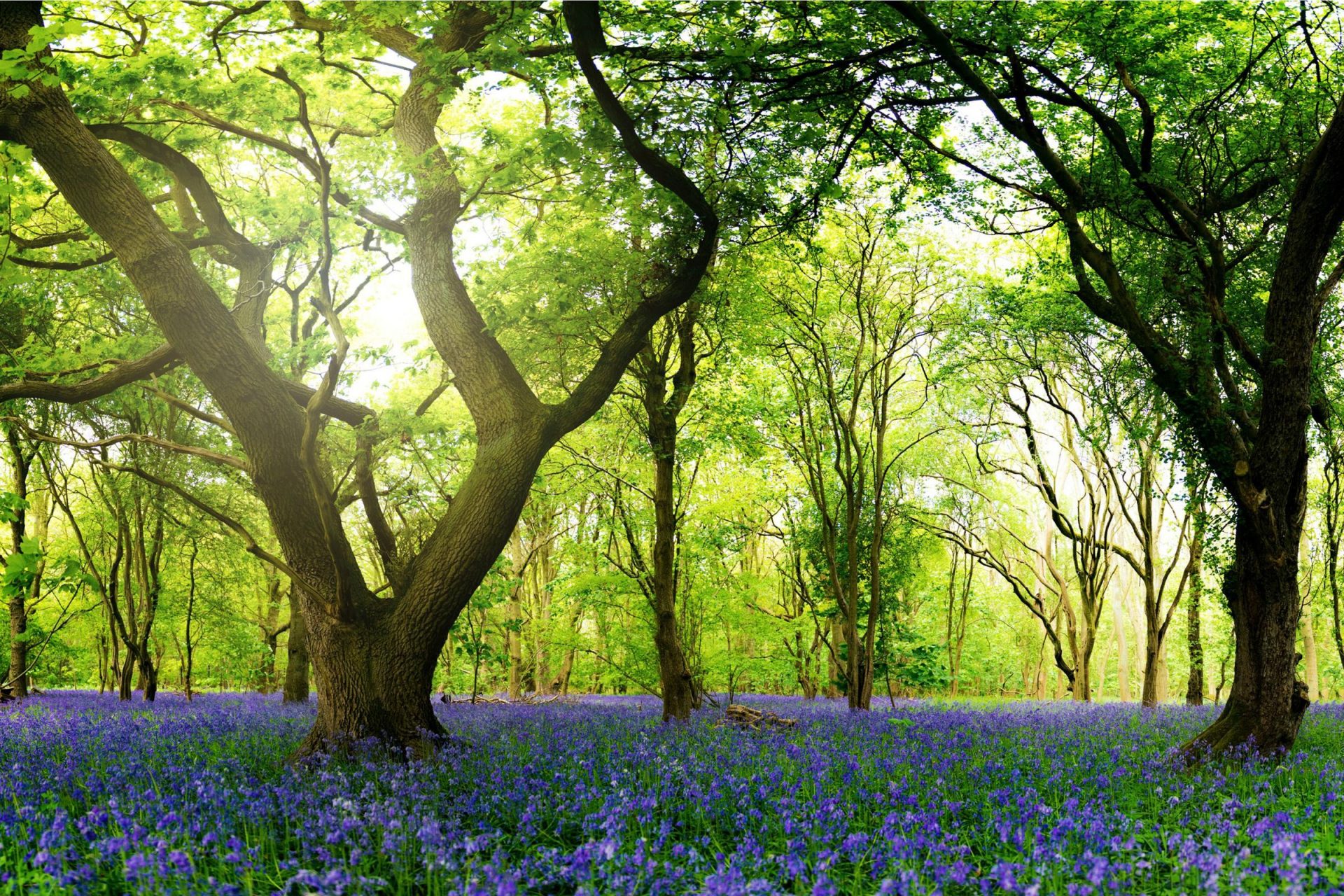
Plants and forests, as well as oceans and landscapes of all kinds, have kept Earth’s natural carbon cycle in balance since long before humans even stood upright. Forests are still the earth’s primary means of absorbing CO2 from the atmosphere today, and their continued productivity is a key factor in global routes to net zero.
BECCS and negative emissions, which will be essential to reach net zero, can only be achieved if there is an abundance of remaining healthy, productive sustainable forests capturing CO2 to lock away permanently.
For large-scale decarbonisation to be successful, countries and companies have to change their relationship with the earth. It is essential that global dependency on fossil fuels is reduced, leaving this pre-historic carbon in the ground and out of the carbon cycle.
“We’re trying to ensure that our learnings in both biomass and CCUS will be transferable,” says Clayton. “We can help the world’s economies create their own negative emissions technologies and projects, making sure that sustainability is at the top of the agenda as the world generates energy and deploys its resources in the future.”